Neurons: Where does their electricity come from?
Tired of struggling to learn neuron action potentials?
Where do neurons get their electricity? Is electricity of the nervous system anything like the household electricity used by light bulbs? To begin the story of neuron action potentials and neuron electricity, let’s talk first about neuron anatomy.
Neuron anatomy
How do neuron cell illustrations differ from real neuron anatomy? It is common practice in teaching human anatomy to use drawings like the one above of a multipolar neuron. It is good to remember, though, that illustrations of neurons lack much detail of the real cells.
In the central nervous system, brain and spinal cord, neurons are packed closely together. The staining method used to capture this photomicrograph only marked 1%-3% of the neurons present. Yet, the photo seems crowded.
Engineers modeled the fluid filled space around brain neurons and described it as a network of pores and tunnels less than 100 nanometers across. A nanometer is 1 part of a meter that is divided into one billion equal parts. Such tight cellular packing creates a setting where chemical communication occurs across very small spaces. This favors the use of diffusion of molecules for communication purposes as you will see as you continue reading.
Neuron electricity
Neuron electricity is carried by ions traveling through neuron cell membranes. Electrical current in physiology consists of a stream of atoms called ions. Ions possess either a positive or a negative electrical charge. The quality and quantity of an ion’s charge is governed by the lack of a match between its number of protons (positive particles) and electrons (negative particles).
The difference between the ion concentration of the fluid in the small spaces surrounding neurons and the ion concentration of the neuron’s cytoplasm drives the flow of ions across the cell membrane when there are open holes in the membranes called ion channels.
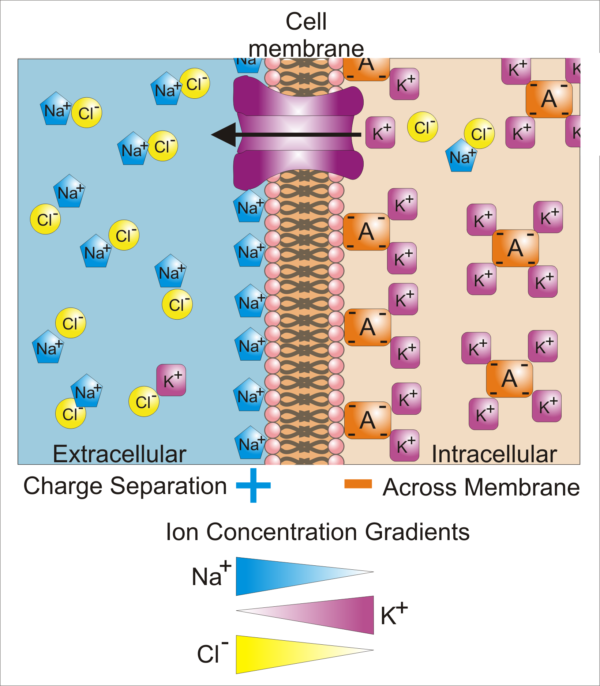
Difference in cellular ion concentrations and those of the extracellular fluid, Synaptidude/WikiMedia Commons
This illustration shows a cell membrane with an open potassium ion [K+] channel. All cell plasma membranes have a small number of open, or leaky, K+ channels that create a transmembrane potential. The leak of the K+ that normally balances the negatively charged intracellular proteins leaves the inside of the cell membrane more negative than the outside surface of the cell membrane. Notice in the illustration the positive sodium ions [Na+] lining the extracellular surface of the cell membrane and the negatively charged proteins lining its intracellular surface.
Transmembrane potential
The transmembrane potential is a useful tool to quantify the relative difference in the electrical potential (negative or positive field) of the two sides of a cell membrane. Transmembrane potentials are measured as voltage. By definition, a voltage is a difference in electrical potential between two points.
When the cell membrane’s electrical potential is different on its two surfaces, there is a voltage across the membrane. The voltage across a cell membrane is measured the same way as the voltage of a car battery – only it is done with a microscope and very tiny probes. The voltage across a cell membrane, the transmembrane potential, is expressed in millivolts – one thousandths of a volt.
The transmembrane potential of inactive axons, those not transmitting signals, is called the transmembrane resting potential. The transmembrane resting potential of most neuron axons measures in the range of -60 to -90 millivolts. By convention in physiology, the positive or negative quality of the transmembrane potential is always stated as the electrical potential of the intracellular surface of the membrane relative to the electrical potential of the outside surface of the membrane.
Voltage-gated ion channels
Neurons use ion channels that open and close based upon the status of the transmembrane potential. These are called voltage-gated ion channels. For electrical signaling by a neuron’s axon, the important voltage-gated ion channels are those for Na+, and K+. When a neuron is at rest the K+ and Na+ channels are closed, and electrical current does not flow.
Ion channels are proteins embedded in the plasma membrane. Proteins are made of 20 unique molecular building blocks called amino acids strung together in a long chain.
Because of the different size of, and charge carried by, individual amino acids protein chains fold over on themselves. In doing so each amino acid searches for space and a comfortable local electrical environment. When there is a change in the surrounding electrical environment a protein must readjust its amino acids and therefore its shape.
In one electrical environment a protein may form an effective barrier between the fluid inside and outside the cell. In another electrical environment the same protein may open up a channel that allows ions to flow through it into the fluid on the opposite side of the membrane.
For neuron axons, certain changes in the electrical field of their membrane surfaces, that is changes in the transmembrane potential, force membrane ion channel proteins into an open state. While all cell membranes have an electrical field on their inside and outside surface, not all cells have proteins that open a hole in the membrane when the electrical field changes.
Neuron action potential
A neuron action potential is a pattern of voltage transients, a short-lived and rapid fluctuation in the transmembrane potential. The rapid fluctuation is caused by opening and closing of voltage-gated ion channel proteins for Na+ and K+.
Voltage sensitive Na+ and K+ protein channels are closed to the flow of ions at the axon’s negative transmembrane resting potential. When the transmembrane potential moves away from its negative resting value to a sufficiently less negative voltage called threshold, the Na+ channel proteins shift their amino acid configuration into an open state.
The move of the transmembrane potential to threshold is caused by an increase in intracellular Na+ from a nearby intracellular source. A positive change in transmembrane potential at the initial segment of the axon is set in motion by an increase in Na+ within the neuron’s cell body.
When a protein channel is open, it allows ions to flow from the fluid where they are in high concentration to the fluid where they are in low concentration. There are separate voltage-gated channel proteins for Na+ and K+. The channel for Na+ opens first when the transmembrane potential reaches threshold positivity. This allows Na+ to flow into the cell where its concentration is still relatively low compared to its concentration in the fluid surrounding the neuron.
The entry of Na+ makes the electrical potential on the inside of the membrane less negative than before the channel opened. This moves the transmembrane potential further from its negative resting value. The change of the transmembrane potential to a high positive value eventually opens the K+ voltage-sensitive channel proteins. Potassium ion then flows out of the cell because the extracellular fluid is low in K+.
The loss of K+ positive charge drives the transmembrane potential back toward its resting negative value. As the transmembrane potential drops, the Na+ channel closes and the flow of Na+ stops. When the transmembrane potential is sufficiently negative, the K+ channel also closes. Notice that the axon’s action potential, the entire cycle of fluctuation of the transmembrane potential takes only 2-3 milliseconds.
Neuron conduction
Probably the most difficult part of understanding neuron electricity is to figure out neuron conduction, how action potentials move from the initial end of the axon near the cell body to the axon’s terminal. The key to understanding this is to recognize that when Na+ enters the cell during an action potential, it diffuses within the axon away from its ion channel location in all directions.
Some of the diffusing Na+ travels toward the terminal end of the axon, away from the cell body. This excess of intracellular Na+ makes the transmembrane potential of the next segment of axon membrane more positive. The Na+ channels of the next segment open initiating another transient action potential a little further along.
Intracellular Na+ diffusing back toward the cell body cannot depolarize the transmembrane potential enough to re-open Na+ channels there, because the K+ channels are still open finishing up the reversal of the previous action potential.
The sequence of ion channels locations along the axon, and the sequence in which the ion channels open and close, ensure that once an action potential is triggered by the cell body, further action potentials can only proceed along the axon in one direction, from the cell body to the axon terminal.
While action potentials proceed in only one direction, the back flow of diffusing ions through the axon is also important for informing the neuron cell body of the level of activity in the axon. Therefore, neuron electricity is not an unimpeded flow of chemical electricity along the axon’s length. Rather it is a series of transmembrane potential alterations that open voltage sensitive ion channels across discrete sections of the axon membrane.
The series of transient changes in transmembrane potential proceed sequentially along the axon membrane from the cell body to the axon terminal. When the depolarization of the transmembrane potential reaches the axon terminal it sets in motion an entirely different type of voltage gated ion channels. That sequence of events is discussed in another article ‘Brain Synapses’. By contrast, household electricity moves along the length of a copper wire flowing in only one direction driven by the potential difference between the ends of the wire.
Do you have questions?
This can be a confusing subject. Please put your questions in the comment box or send me an email at DrReece@MedicalScienceNavigator.com. I read and reply to all comments and email.
If you think this description of action potentials is helpful, share it with your fellow students or send it to your favorite social media by clicking one of the buttons below.
Further reading:
Margaret Thompson Reece PhD, physiologist, former Senior Scientist and Laboratory Director at academic medical centers in California, New York and Massachusetts is now Manager at Reece Biomedical Consulting LLC.
She taught physiology for over 30 years to undergraduate and graduate students, at two- and four-year colleges, in the classroom and in the research laboratory. Her books “Physiology: Custom-Designed Chemistry”, “Inside the Closed World of the Brain”, and her online course “30-Day Challenge: Craft Your Plan for Learning Physiology”, and “Busy Student’s Anatomy & Physiology Study Journal” are created for those planning a career in healthcare. More about her books is available at https://www.amazon.com/author/margaretreece. You may contact Dr. Reece at DrReece@MedicalScienceNavigator.com, or on LinkedIn.
Dr. Reece offers a free 30 minute “how-to-get-started” phone conference to students struggling with human anatomy and physiology. Schedule an appointment by email at DrReece@MedicalScienceNavigator.com.
very useful information …
off to share
Thank you.
I wonder what will happen if we transmitted a 1 voltage houseelectricity to a neuron? Will it destroy the neuron or will the neuron transmit it to the brain?
It would probably kill the neuron. Your body is mostly water. Water transmits house electricity very well. Do not mess with house electricity. It has been known to kill people.